Computer-assisted surgery: Looking to the future
Preview
It can take 20 years for a new medical technology to mature through the various development stages. In a way, today’s “cutting-edge” technology reflects the ideas of yesterday. Part 3 gazes into the future of CAS. Will virtual reality rule the operating room? Will there still be a need for a human surgeon?
Computer-assisted surgery (CAS) systems, such as navigation and surgical robots, are currently available in select operating rooms (ORs) around the world. Part 1 introduced the terminology used in CAS and the different types of CAS technology being used. Part 2 looked at the evidence for and against the use of computer-assisted orthopedic surgery (CAOS) systems, concluding that more studies—particularly related to long-term clinical outcomes—are needed to determine the impact of these technologies beyond the documented improved surgical/implant placement accuracy, especially in terms of reconciling costs.
So what direction is technology taking the field of CAS in orthopedics?
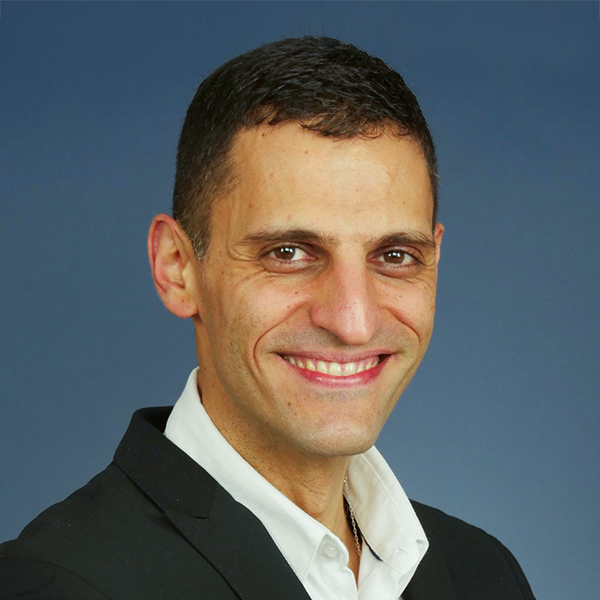
Mark Roussot
University College London Hospital
London, United Kingdom
“The next frontier in hip and knee surgery may include advancements in joint preservation, soft tissue reconstruction, tissue engineering, and training,” says Mark Roussot, MBChB MPhil, MMed, FC Orth, of the University College London Hospital, Department of Trauma and Orthopaedics.
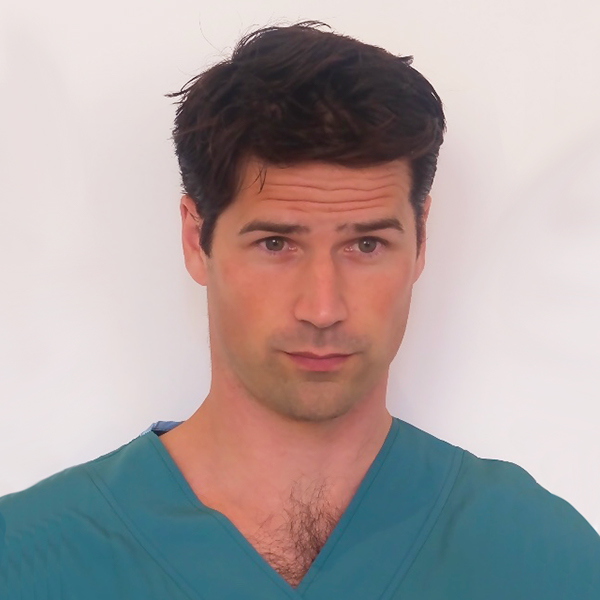
Georges Vles
University Hospitals Leuven
Leuven, Belgium
Georges Vles, MD, PhD, from Belgium’s University Hospitals Leuven, Division of Orthopedic Surgery thinks that, “For me as a hip surgeon, I think the next frontier would be robot-assisted CAM resection. For knees, the logical next steps would be osteotomies (getting a perfect angle and always leaving the hinge intact) and ACL [anterior cruciate ligament] reconstruction (bone tunnels and graft preloading and tensioning).”
Let us look at some of the enduring challenges in orthopedics that have great potential to be resolved with the CAS systems of the future.
Surgeon training
Virtual reality (VR) training for surgeons is still in its infancy and the financial investment of a VR simulation system can be significant, depending on its complexity [1,2]. Mark Roussot points out that, “Virtual reality, augmented reality, and procedures performed with CAS/robots on cadavers provide safe environments for trainees to rehearse procedural steps, become accustomed to the technology, and learn about the nuances of achieving a well-aligned, well-balanced, and stable joint replacement. Like other industries, simulation-based training will become increasingly utilized.”
One concern that has been raised around computer-based training is the establishment of proficiency standards. If, after a certain number of attempts or time, simulation training ends, it does not take into account the large variation in attempts individuals take to learn a procedure [3]. See Figure 1. To be effective, VR training should differentiate between user experience levels, then tailor the training accordingly. Interestingly, how precisely a simulation replicates what it is modeling does not necessarily produce superior training outcomes—a low-fidelity laparoscopy simulator was shown to be superior to a comparable high-fidelity simulator in skills transfer to the OR [4].
Augmented reality
Augmented reality (AR) is another modality that has potential to train surgeons and offer guidance during procedures. AR incorporates dynamic VR elements and merges them in real time within a user’s perceived visual environment [5]. For example, it might allow a local surgeon to see an image of a remote surgeon’s hands within their field of view during an actual surgery, or images of a patient’s hidden anatomy superimposed over their field of view. See Figure 2.
Use of AR for surgeon training is not widespread, however, the AR interface has been explored in more depth in relation to distance medicine.
Telemedicine
Providing high-quality medical care and specialist consultation to remote communities has several challenges, including high costs and inconvenience related to travel. Real-time video conferencing between geographically separated orthopedic specialists and patients was shown to offer cost savings [6, 7] and access to care otherwise not easily found [8]. A 2019 study allowed general practitioners to evaluate the cases of highly rural patients collaboratively with remote orthopedic specialists, allowing nearly 70% of patients’ issues to be resolved during this first evaluation. Ultimately, for those who required a referral, the wait time was reduced from 201 to 40 days [9].
Read the full article with your AO login
- Telepresence
- Rehabilitation video games
- Knee ligament reconstruction
- Joint preservation
- Periacetabular osteotomies
- Hip arthroscopy
- Spine
- Shoulders
- Tumors
- Trauma: Fracture reduction
- Hip and knee arthroplasty
- Future evolution of CAS
- Outside the box thinking: collaboration to drive change
- Open and closed systems
- Machine communication and interaction
- Who determines the parameters?
- Will robots make surgeons irrelevant?
- Conclusion
- References
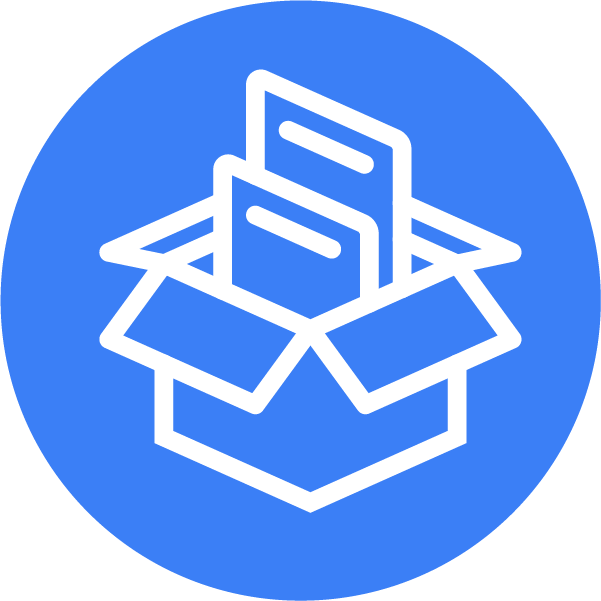
Additional AO resources on this topic
Access videos, tools, and other assets to learn more about this topic.
- Video: Robotics and Other Smart Tools for Hip and Knee Replacement
- Further reading: The development and validation of a robotic system for sacroiliac luxation/fracture reduction and fixation
- Upcoming events: AO Recon Course finder
Contributing experts
This series of articles was created with the support of the following specialists (in alphabetical order):
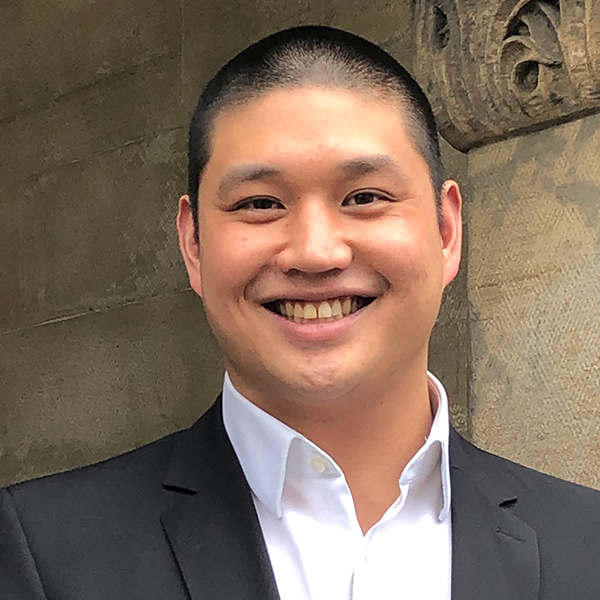
Justin Chang
University College London Hospital
London, United Kingdom
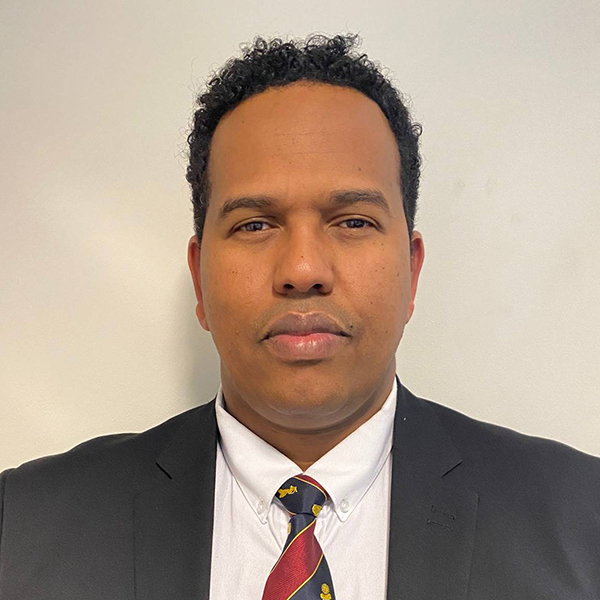
Ahmed Magan
University College London Hospital
London, United Kingdom
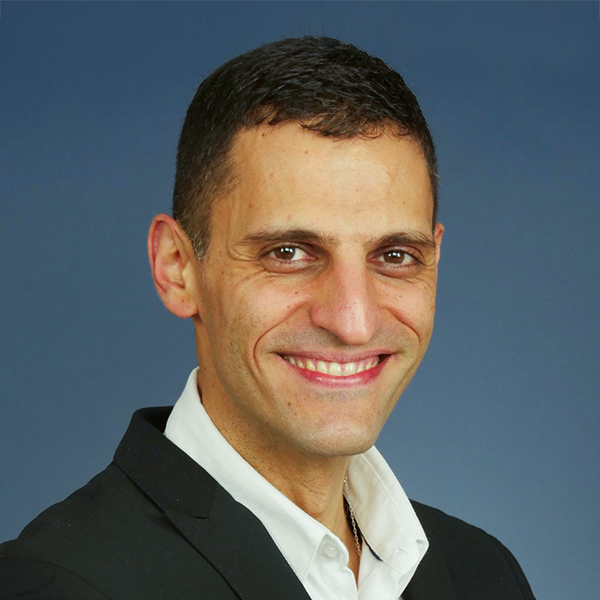
Mark Roussot
University College London Hospital
London, United Kingdom
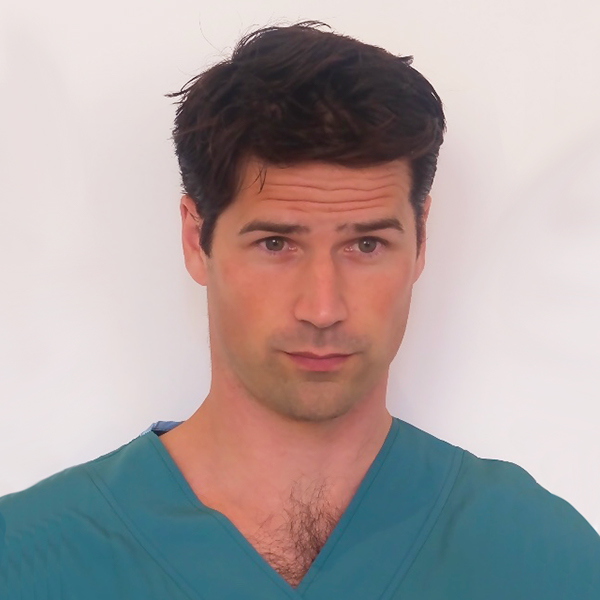
Georges Vles
University Hospitals Leuven
Leuven, Belgium
This issue was created by Word+Vision Media Productions, Switzerland
References
- Willy C. [Simulation techniques in surgical training programs to become an orthopedic and trauma surgeon : Introduction to the topic]. Unfallchirurg. 2019 Jun;122(6):418–424.
- Sheik-Ali S, Edgcombe H, Paton C. Next-generation Virtual and Augmented Reality in Surgical Education: A Narrative Review. Surg Technol Int. 2019 Nov 10;35:27–35.
- Gustafsson A, Pedersen P, Rømer TB, et al. Hip-fracture osteosynthesis training: exploring learning curves and setting proficiency standards. Acta Orthop. 2019 Aug;90(4):348–353.
- Tan SC, Marlow N, Field J, et al. A randomized crossover trial examining low- versus high-fidelity simulation in basic laparoscopic skills training. Surg Endosc. 2012 Nov;26(11):3207–3214.
- Baker D, Fryberger C, Ponce B. The Emergence of Augmented Reality in Orthopaedic Surgery and Education. The Orthopaedic Journal at Harvard Medical School. 2015 June;16.
- Buvik A, Bergmo TS, Bugge E, et al. Cost-Effectiveness of Telemedicine in Remote Orthopedic Consultations: Randomized Controlled Trial. J Med Internet Res. 2019 Feb 19;21(2):e11330.
- Aponte-Tinao LA, Farfalli GL, Albergo JI, et al. Face to Face Appointment vs. Telemedicine in First Time Appointment Orthopedic Oncology Patients: A Cost Analysis. Stud Health Technol Inform. 2019 Aug 21;264:512–515.
- Lese A, Sraj S. Rural Orthopedics: Providing Orthopedic Care in Rural Communities. Orthopedics. 2019 Jul 1;42(4):e350–e355.
- Prada C, Izquierdo N, Traipe R, et al. Results of a New Telemedicine Strategy in Traumatology and Orthopedics. Telemed J E Health. 2019 Jul 9.
- Shenai MB, Dillavou M, Shum C, et al. Virtual interactive presence and augmented reality (VIPAR) for remote surgical assistance. Neurosurgery. 2011 Mar;68(1 Suppl Operative):200–207.
- Marescaux J, Leroy J, Gagner M, et al. Transatlantic robot-assisted telesurgery. Nature. 2001 Sept;413(6854):379–380.
- Garcia P, Rosen J, Kapoor C, et al. Trauma Pod: a semi-automated telerobotic surgical system. Int J Med Robot. 2009 Jun;5(2):136–146.
- Emerging Technology from the arXivarchive page. Security Experts Hack Teleoperated Surgical Robot. 2015. https://www.technologyreview.com/2015/04/24/168339/security-experts-hack-teleoperated-surgical-robot/. Accessed May 3, 2020.
- Lohse K, Shirzad N, Verster A, et al. Video games and rehabilitation: using design principles to enhance engagement in physical therapy. J Neurol Phys Ther. 2013 Dec;37(4):166–175.
- Janssen J, Verschuren O, Renger WJ, et al. Gamification in Physical Therapy: More Than Using Games. Pediatr Phys Ther. 2017 Jan;29(1):95–99.
- Novak D, Nagle A, Keller U, et al. Increasing motivation in robot-aided arm rehabilitation with competitive and cooperative gameplay. J Neuroeng Rehabil. 2014 Apr 16;11:64.
- Padilla-Castañeda MA, Sotgiu E, Barsotti M, et al. An Orthopaedic Robotic-Assisted Rehabilitation Method of the Forearm in Virtual Reality Physiotherapy. J Healthc Eng. 2018 2018:7438609.
- Dessenne V, Lavallée S, Julliard R, et al. Computer-assisted knee anterior cruciate ligament reconstruction: first clinical tests. J Image Guid Surg. 1995 1(1):59–64.
- Cho WJ, Kim JM, Kim DE, et al. Accuracy of the femoral tunnel position in robot-assisted anterior cruciate ligament reconstruction using a magnetic resonance imaging-based navigation system: A preliminary report. Int J Med Robot. 2018 Oct;14(5):e1933.
- Śmigielski R, Zdanowicz U, Drwięga M, et al. The anatomy of the anterior cruciate ligament and its relevance to the technique of reconstruction. Bone Joint J. 2016 Aug;98-b(8):1020–1026.
- Okoroha KR, Evans TJ, Stephens JP, et al. Three-dimensional printing improves osteochondral allograft placement in complex cases. Knee Surg Sports Traumatol Arthrosc. 2018 Dec;26(12):3601–3605.
- Abdulghani S, Morouço PG. Biofabrication for osteochondral tissue regeneration: bioink printability requirements. J Mater Sci Mater Med. 2019 Jan 28;30(2):20.
- Yamaguchi KT, Cheung EC, Markolf KL, et al. Effects of Anterior Closing Wedge Tibial Osteotomy on Anterior Cruciate Ligament Force and Knee Kinematics. Am J Sports Med. 2018 Feb;46(2):370–377.
- Vap AR, Schon JM, Moatshe G, et al. The Role of the Peripheral Passive Rotation Stabilizers of the Knee With Intact Collateral and Cruciate Ligaments: A Biomechanical Study. Orthop J Sports Med. 2017 May;5(5):2325967117708190.
- Song EK, Seon JK, Yim JH, et al. Robotic-assisted TKA reduces postoperative alignment outliers and improves gap balance compared to conventional TKA. Clin Orthop Relat Res. 2013 Jan;471(1):118–126.
- Kim HJ, Yoon JR, Choi GW, et al. Imageless Navigation Versus Conventional Open Wedge High Tibial Osteotomy: A Meta-Analysis of Comparative Studies. Knee Surg Relat Res. 2016 Mar;28(1):16–26.
- Wikipedia. Goniometer. Published 2020. Updated April 13, 2020. Accessed May 2, 2020.
- Liu L, Ecker T, Schumann S, et al. Computer assisted planning and navigation of periacetabular osteotomy with range of motion optimization. Med Image Comput Comput Assist Interv. 2014 17(Pt 2):643–650.
- Hooper JM, Mays RR, Poultsides LA, et al. Periacetabular osteotomy using an imageless computer-assisted navigation system: a new surgical technique. J Hip Preserv Surg. 2019 Dec;6(4):426–431.
- Langlotz F, Stucki M, Bächler R, et al. The first twelve cases of computer assisted periacetabular osteotomy. Comput Aided Surg. 1997 2(6):317–326.
- Wirth SH, Rahm S, Kamath AF, et al. Periacetabular osteotomy using three-dimensional cutting and reposition guides: a cadaveric study. Journal of Hip Preservation Surgery. 2019 6(4):411–420.
- Wylie JD, Ferrer MG, McClincy MP, et al. What Is the Reliability and Accuracy of Intraoperative Fluoroscopy in Evaluating Anterior, Lateral, and Posterior Coverage During Periacetabular Osteotomy? Clin Orthop Relat Res. 2019 May;477(5):1138–1144.
- Anderson L, Erickson J, Peters C. Validation of a quantitative fluoroscopic tool with distortion correction for acetabular positioning in periacetabular osteotomy. The Hip Society (THS) 2019 Summer Meeting; 2019; Kohler, WI, USA,.
- Kobayashi N, Inaba Y, Kubota S, et al. Computer-Assisted Hip Arthroscopic Surgery for Femoroacetabular Impingement. Arthrosc Tech. 2018 Apr;7(4):e397–e403.
- Van Houcke J, Khanduja V, Nakano N, et al. Accuracy of navigated cam resection in femoroacetabular impingement: A randomised controlled trial. Int J Med Robot. 2017 Dec;13(4).
- Ghasem A, Sharma A, Greif DN, et al. The Arrival of Robotics in Spine Surgery: A Review of the Literature. Spine (Phila Pa 1976). 2018 Dec 1;43(23):1670–1677.
- Vardiman AB, Wallace DJ, Crawford NR, et al. Pedicle screw accuracy in clinical utilization of minimally invasive navigated robot-assisted spine surgery. J Robot Surg. 2019 Jul 19.
- Smith JS, Shaffrey CI, Ames CP, et al. Treatment of adult thoracolumbar spinal deformity: past, present, and future. J Neurosurg Spine. 2019 May 1;30(5):551–567.
- Villatte G, Muller AS, Pereira B, et al. Use of Patient-Specific Instrumentation (PSI) for glenoid component positioning in shoulder arthroplasty. A systematic review and meta-analysis. PLoS One. 2018 13(8):e0201759.
- So TY, Lam YL, Mak KL. Computer-assisted navigation in bone tumor surgery: seamless workflow model and evolution of technique. Clin Orthop Relat Res. 2010 Nov;468(11):2985–2991.
- Farfalli GL, Albergo JI, Ritacco LE, et al. What Is the Expected Learning Curve in Computer-assisted Navigation for Bone Tumor Resection? Clin Orthop Relat Res. 2017 Mar;475(3):668–675.
- Nandra R, Matharu G, Stevenson J, et al. Long-term outcomes after an initial experience of computer-navigated resection of primary pelvic and sacral bone tumours: soft-tissue margins must be adequate to reduce local recurrences. Bone Joint J. 2019 Apr;101-b(4):484–490.
- Hollon TC, Pandian B, Adapa AR, et al. Near real-time intraoperative brain tumor diagnosis using stimulated Raman histology and deep neural networks. Nat Med. 2020 Jan;26(1):52–58.
- Bai L, Yang J, Chen X, et al. Medical Robotics in Bone Fracture Reduction Surgery: A Review. Sensors (Basel). 2019 Aug 18;19(16).
- Oszwald M, Westphal R, Stier R, et al. Hands-on robotic distal interlocking in intramedullary nail fixation of femoral shaft fractures. Technol Health Care. 2010 18(4-5):325–334.
- Gosling T, Westphal R, Hufner T, et al. Robot-assisted fracture reduction: a preliminary study in the femur shaft. Med Biol Eng Comput. 2005 Jan;43(1):115–120.
- Lei H, Sheng L, Manyi W, et al. A biplanar robot navigation system for the distal locking of intramedullary nails. Int J Med Robot. 2010 Mar;6(1):61–65.
- Wang JQ, Wang Y, Feng Y, et al. Percutaneous Sacroiliac Screw Placement: A Prospective Randomized Comparison of Robot-assisted Navigation Procedures with a Conventional Technique. Chin Med J (Engl). 2017 Nov 5;130(21):2527–2534.
- Duan SJ, Liu HS, Wu WC, et al. Robot-assisted Percutaneous Cannulated Screw Fixation of Femoral Neck Fractures: Preliminary Clinical Results. Orthop Surg. 2019 Feb;11(1):34–41.
- Wang XD, Lan H, Li KN. Treatment of Femoral Neck Fractures with Cannulated Screw Invasive Internal Fixation Assisted by Orthopaedic Surgery Robot Positioning System. Orthop Surg. 2019 Oct;11(5):864–872.
- Lan H, Tan Z, Li KN, et al. Intramedullary Nail Fixation Assisted by Orthopaedic Robot Navigation for Intertrochanteric Fractures in Elderly Patients. Orthop Surg. 2019 Apr;11(2):255–262.
- Liu B, Wu F, Chen S, et al. Robot-assisted percutaneous scaphoid fracture fixation: a report of ten patients. J Hand Surg Eur Vol. 2019 Sep;44(7):685–691.
- Zhao JX, Li C, Ren H, et al. Evolution and Current Applications of Robot-Assisted Fracture Reduction: A Comprehensive Review. Ann Biomed Eng. 2020 Jan;48(1):203–224.
- Buschbaum J, Fremd R, Pohlemann T, et al. Computer-assisted fracture reduction: a new approach for repositioning femoral fractures and planning reduction paths. Int J Comput Assist Radiol Surg. 2015 Feb;10(2):149–159.
- Dagnino G, Georgilas I, Köhler P, et al. Navigation system for robot-assisted intra-articular lower-limb fracture surgery. Int J Comput Assist Radiol Surg. 2016 Oct;11(10):1831–1843.
- Oszwald M, Westphal R, Bredow J, et al. 3D visualized robot assisted reduction of femoral shaft fractures: evaluation in exposed cadaveric bones. Technol Health Care. 2009 17(4):337–343.
- Zhu Q, Liang B, Wang X, et al. Minimally invasive treatment of displaced femoral shaft fractures with a teleoperated robot-assisted surgical system. Injury. 2017 Oct;48(10):2253–2259.
- Roussot M, Vles G, Oussedik S. Clinical outcomes of kinematic alignment versus mechanical alignment in total knee arthroplasty – a systematic review. [Accepted for publication]. EFORT Open Reviews. 2020.
- Oussedik S, Abdel MP, Victor J, et al. Alignment in total knee arthroplasty. Bone Joint J. 2020 Mar;102-b(3):276–279.
- Ulivi M, Orlandini LC, Meroni V, et al. Intraoperative validation of bone cut accuracy of a pinless smart touch-screen navigation system device in total knee arthroplasty. Int J Med Robot. 2019 Oct;15(5):e2030.
- Shademan A, Decker RS, Opfermann JD, et al. Supervised autonomous robotic soft tissue surgery. Sci Transl Med. 2016 May 4;8(337):337ra364.
- Jones LD, Golan D, Hanna SA, et al. Artificial intelligence, machine learning and the evolution of healthcare: A bright future or cause for concern? Bone Joint Res. 2018 Mar;7(3):223–225.
- Bayliss L, Jones LD. The role of artificial intelligence and machine learning in predicting orthopaedic outcomes. Bone Joint J. 2019 Dec;101-b(12):1476–1478.
- Ungi T, Lasso A, Fichtinger G. Open-source platforms for navigated image-guided interventions. Med Image Anal. 2016 Oct;33:181–186.
- Frank T, Krieger A, Leonard S, et al. ROS-IGTL-Bridge: an open network interface for image-guided therapy using the ROS environment. Int J Comput Assist Radiol Surg. 2017 Aug;12(8):1451–1460.
- Arata J, Kozuka H, Kim HW, et al. Open core control software for surgical robots. Int J Comput Assist Radiol Surg. 2010 May;5(3):211–220.
- Applied Dexterity. Applied Dexterity: Driving innovation in surgical robotics. http://applieddexterity.com/ Published 2020. Accessed May 3, 2020.
- Hannaford B, Rosen J, Friedman DW, et al. Raven-II: an open platform for surgical robotics research. IEEE Trans Biomed Eng. 2013 Apr;60(4):954–959.
- Lopez E, Kwok KW, Payne CJ, et al. Implicit Active Constraints for Robot-Assisted Arthroscopy. IEEE Int Conf Robot Autom. 2013 May 10;2013:5390–5395.
- Dhawan A, Kennedy PM, Rizk EB, et al. Three-dimensional Bioprinting for Bone and Cartilage Restoration in Orthopaedic Surgery. J Am Acad Orthop Surg. 2019 Mar 1;27(5):e215–e226.
- Xu T, Binder KW, Albanna MZ, et al. Hybrid printing of mechanically and biologically improved constructs for cartilage tissue engineering applications. Biofabrication. 2013 Mar;5(1):015001.
- Shim JH, Jang KM, Hahn SK, et al. Three-dimensional bioprinting of multilayered constructs containing human mesenchymal stromal cells for osteochondral tissue regeneration in the rabbit knee joint. Biofabrication. 2016 Feb 4;8(1):014102.
- Pirlich M, Stöhr M, Neumuth T, et al. The Intelligent ENT Operating Room of the Future. Laryngorhinootologie. 2019 Mar;98(S 01):S5–S31.
- Jarvis C. Opinion: Surgical Robots are Surging in Popularity. So Will Their Data. Undark. https://undark.org/2019/08/15/surgical-robots-are-suring-in-popularity/. Published 2019. Updated August 15, 2019. Accessed May 3, 2020.
- World Bank Group. The Changing Nature of Work. 2019:10.
- International Federation of Robotics. Facts about robots. https://ifr.org/. Published 2020. Accessed May 3, 2020.
- Boyle A. Why your robotic surgeon will have a human overlord looking over its shoulder. GeekWire. https://www.geekwire.com/2016/robot-surgeon-human-overlord/. Published 2016. Updated April 27, 2016. Accessed May 3, 2020.